Promoting Canine Health and Longevity with Salmon Meals
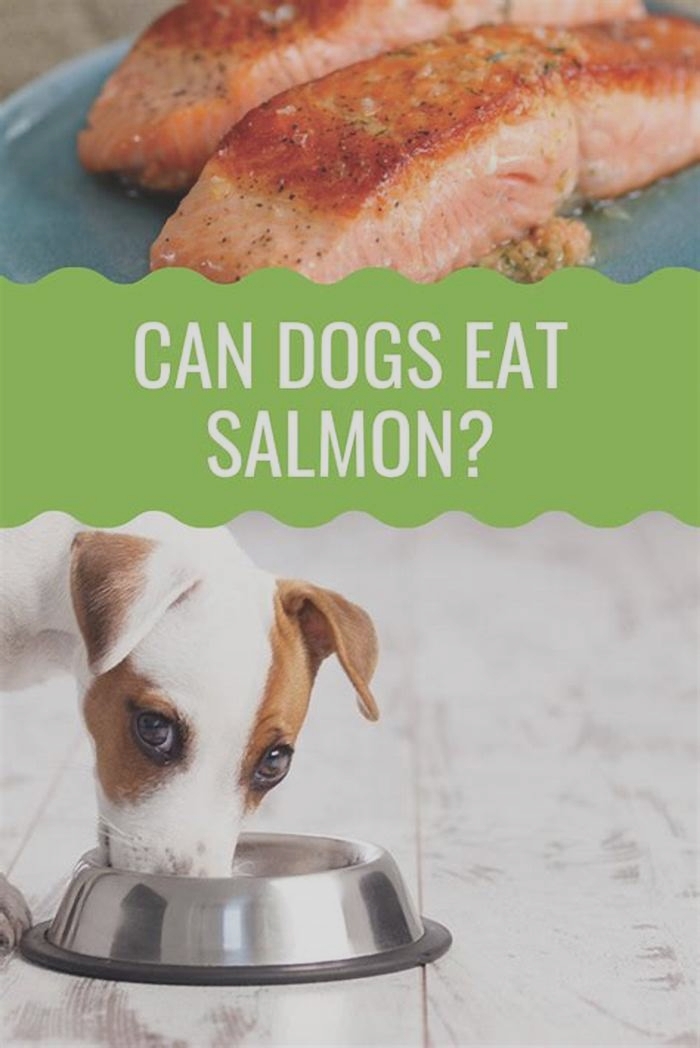
Promoting Health and Longevity through Diet: from Model Organisms to Humans
Introduction
The discovery that aging can be ameliorated by dietary, genetic and pharmacological interventions has opened up the prospect of a broad-spectrum, preventative medicine for aging-related diseases () (Fontana et al., 2014; Goldman et al., 2013; Partridge, 2010). Single gene mutations that extend animal lifespan can ameliorate natural, age-dependent loss of function (Metaxakis et al., 2014; Stein and Murphy, 2012), and the pathology of aging-related diseases, including neurodegeneration (Cohen et al., 2009; Killick et al., 2009; Menzies and Rubinsztein, 2010; Pinkston-Gosse and Kenyon, 2007; Stohr et al., 2013). Furthermore, laboratory animal models of slowed aging, naturally long-lived species such as the naked mole rat, and some humans that achieve the age of 100, have all demonstrated that a long life is not inevitably associated with late-life disability and disease (Ikeno et al., 2006; Edrey et al., ILAR J 2011; Ailshire et al., JG 2014). Recent work has shown that specific dietary interventions can also promote long life and healthy old age.
Tab. 1
Interventions extending mean or/and maximal lifespan in wild type mice fed normal chow
Max lifespan | Mean lifespan | Main mechanism of action | |
---|---|---|---|
Dietary interventions | |||
Calorie restriction | Yes | Yes | nutrient sensing pathways |
Intermittent fasting | Yes | Yes | nutrient sensing pathways |
Protein restriction | No | Yes | nutrient sensing pathways |
Methionine restriction | Yes | Yes | nutrient sensing pathways |
Tryptophan restriction | Yes | Yes | nutrient sensing pathways |
Physical exercise interventions | |||
Endurance exercise | No | Yes | insulin sensitivity; AMPK ? |
Genetic interventions | |||
Ames and Snell dwarf | Yes | Yes | nutrient sensing pathways |
GH receptor KO | Yes | Yes | nutrient sensing pathways |
IGF-1 R KO | Yes (in F) | Yes (in F) | nutrient sensing pathways |
Klotho TG | Yes (in M) | Yes | nutrient sensing pathways |
Fat Insulin Receptor KO | Yes | Yes | nutrient sensing pathways |
Insulin Receptor Substrate 1 KO | Yes (only F) | Yes (only F) | nutrient sensing pathways |
Brain IRS-2 KO | Yes | Yes | nutrient sensing pathways |
PAPP-A KO | Yes | Yes | nutrient sensing pathways |
Ribosomal S6 protein kinase-1 KO | Yes (only F) | Yes (only F) | nutrient sensing pathways |
FGF-21TG | Yes | Yes | nutrient sensing pathways |
miR-17TG | ? | Yes | nutrient sensing pathways |
DGAT1KO | Yes (only F) | Yes (only F) | nutrient sensing pathways |
p66shc KO | Yes | Yes | growth factor mediated signaling |
ATG5 TG | Yes | Yes | autophagy |
Type 5 Adenylyl Cyclase KO | Yes | Yes | -adrenergic signaling |
Angiotensin II type 1 receptor KO | Yes | Yes | angiotensin receptor signaling |
Catalase targeted to mitochondria TG | Yes | Yes | oxidative stress (mitochondrial) |
Ink4/Arf-TG/TG | No | Yes | mitogenic over-stimulation & cell proliferation |
C/EBP / | Yes | Yes | mitochondrial biogenesis in white fat cells |
Mclk1KO | Yes | Yes | age-dependent loss of mitochondrial function |
Hcrt-UCP2 TG | No | Yes | ?; core body temperature |
Macrophage migration inhibitory factor KO | Yes | Yes | inflammation; insulin pathway |
E-DNIB TG | ? | Yes | inflammation; insulin pathway |
PKA RII KO | Yes | Yes | IGF signaling ? |
RasGRF1 KO | Yes | Yes | nutrient sensing pathways |
Sirt6 TG | Yes (only M) | Yes (only M) | nutrient sensing pathways (IGF) |
Brain specific Sirt1 TG | Yes (only F) | Yes | mitochondrial function via Sirt1/Nkx2-1/Ox2r |
TRPV1 pain receptor KO | Yes (only F) | Yes | Modulation of CRTC1/CREB activity |
Pharmacological interventions | |||
Rapamycin | Yes | Yes | nutrient sensing pathways (mTOR) |
Acarbose | Yes | Yes | IGF signaling & FGF-21 |
Metformin | No | Yes | nutrient sensing pathways (AMPK) |
Aspirin | No | Yes (only M) | inflammation |
Nordihydroguaiaretic acid | No | Yes (only M) | inflammation & oxidative stress |
Green tea extract | No | Yes (only F) | oxidative stress |
17-Estradiol (nonfeminizing estrogen) | No | Yes (only M) | ? non genomic actions |
Methylene Blue | No | Yes (only F) | mitochondrial function |
Metoprolol | No | Yes (in M) | -adrenergic receptor signaling |
Nebivolol | No | Yes (in M) | -adrenergic receptor signaling |
Dietary restriction (DR), implemented as chronic and coordinate reduced intake of all dietary constituents except vitamins and minerals, was first shown 80 years ago to extend lifespan in rats. DR in both rats and mice improves most aspects of health during aging (Fontana et al. 2010; Ikeno et al. 2006; Maeda et al., 1985). Exceptions include resistance to infection and wound healing. However, these conditions rapidly improve with re-feeding and DR animals can then outperform controls (Kristan, 2008; Hunt et al., 2012). DR can produce substantial benefits with, for instance, ~30% of DR animals dying at old ages without gross pathological lesions, compared with only 6% of ad-libitum fed controls (Ikeno et al., 2006). DR started in young, adult Rhesus monkeys greatly improves metabolic health, prevents obesity, delays the onset of sarcopenia, presbycusis and brain atrophy, and reduces the risk of developing and dying of type 2 diabetes, cancer and cardiovascular disease (Colman et al., 2014, Mattison et al., 2012).
In humans, severe food restriction without malnutrition results in many of the same physiological, metabolic and molecular changes associated with DR in animals, including less age-associated myocardial stiffness and autonomic dysfunction, lower core body temperature and down-regulation of the PI3K/AKT/FOXO and inflammatory pathways in skeletal muscle (Cava & Fontana. 2013). Furthermore, humans voluntarily undertaking long-term DR score lower than controls on multiple risk factors for cardiovascular disease and cancer (Fontana et al., Handbook, 2010). In short-term, randomized clinical trials in aging humans, DR improves several markers of health (Heilbronn et al., 2006; Fontana et al., Handbook, 2010). However, severe DR with adequate nutrition (i.e. consuming at least 100% of the RDI for each essential nutrient) is not an option for most people, because it is difficult to practice and sustain and, with inadequate nutrition, can increase the risk of impaired menstrual and reproductive function, osteoporotic bone fractures, anemia, and cardiac arrhythmias (Fairburn & Harrison. 2003). Dietary interventions that avoid unrealistic levels of self-deprivation, and pharmacological interventions that recapture beneficial effects of DR, are therefore important goals to improve human health during aging.
DR increases healthy lifespan in many shorter-lived organisms, including budding yeast Saccharomyces cerevisiae, the nematode worm Caenorhabditis elegans and the fruit fly Drosophila melanogaster (). The experimental tractability of yeast and invertebrates facilitates discovery of the, often evolutionarily conserved, mechanisms through which genetic and environmental intervention improve health during aging. The mechanisms mediating the heath benefits of DR are not fully understood in any organism. A wide range of interventions has been used to impose DR, even within single species, and the mechanisms through which they extend lifespan can differ (Bass et al., 2007; Cypser et al., 2013; Mair and Dillin, 2008; Tatar et al., 2014). Multiple neural, systemic, tissue-specific and cell autonomous mechanisms are involved (). In both C. elegans and Drosophila, altered sensory perception alone may play a role, with specific subsets of sensory, including olfactory and gustatory, neurons influencing lifespan (Alcedo and Kenyon, 2004; Allen et al., 2014; Apfeld and Kenyon, 1999; Ostojic et al., 2014; Waterson et al., 2014). In C. elegans, Drosophila and mice, neural circuits both detect nutrient status and control the responses to it, in mice mediated mainly by the hypothalamus (Dacks et al., 2013). Alterations in the levels of metabolites (Chin et al., 2014), in activity of the nutrient-sensing insulin/IGF signaling network (Johnson et al., 2013; Kenyon, 2010) in C. elegans, of steroid hormone signaling (Thondamal et al., 2014) and, in the mouse, of growth hormone (Johnson et al., 2013; Kenyon, 2010) also play important systemic roles. Cellular effector processes can include enhanced genomic stability and chromatin remodeling (Dang et al., 2014), improved chaperone-mediated protein homeostasis and cellular turnover processes including autophagy (Singh and Cuervo, 2012) and increases in various forms of stress resistance (Hine et al., 2014). Molecular effectors that have been shown to mediate the effects of DR on health and longevity include FOXO (Tullet et al., 2008; Webb & Brunet. 2014), TOR (Kapahi et al., 2010; Johnson et al., 2013), AMPK (Geer et al., 2007; Burkewitz et al. 2014), sirtuins (Mouchiroud et al., 2013; Guarente. 2013), HSF and NRF2 (Akerfelt et al., 2010; Martn-Montalvo et al., 2011). Inhibition of AKT activates FOXO, a transcription factor which up-regulates several longevity pathways controlling DNA repair, autophagy, antioxidant activity, and stress resistance, and cell proliferation (Webb & Brunet. 2014; Wang et al., 2014). Inhibition of mTORC1 improves proteostasis, increases autophagy and enhances stem cell function (Kapahi et al., 2010; Efeyan et al. 2012; Johnson et al., 2013). Systemic or tissue specific overexpression of some sirtuins (i.e. SIRT1, SIRT3 and SIRT6) also increases genomic stability, reduces NF-kB signaling, and improves metabolic homeostasis through histone deacetylation (Mouchiroud et al., 2013; Guarente. 2013). Moroever, activation of SIRT1 and AMPK activates PGC-1a, a transcriptional regulator of mitochondrial function, antioxidant defenses, and fatty acid oxidation (Wu et al., 1999). Activation of transcription factors heat shock factor-1 and Nrf2 by upregulating HSP70, p62, and the transcription factor ATF3 induces several antioxidant and drug-metabolizing enzymes, prevents the age-dependent impairment of proteostasis and promotes the maintenance of cell structure, redox, and intermediary metabolism (Akerfelt et al., 2010; Martn-Montalvo et al., 2011). Mutiple, parallel processes thus contribute to the increase in health during aging from DR, and the relative contribution of these may very between DR regimes and organisms.
Dietary restriction increases healthy lifespan in diverse single-celled, invertebrate and vertebrate animals.
Dietary restriction modulates multiple systemic, neural and cellular mechanisms that improve health and combat the diseases of aging.
Interestingly, recent work has revealed the importance of timing of food intake, the role of specific nutrients, the nature of the effector mechanisms, the longer-term, including transgenerational, consequences of diet, and the key role played by the gut microbiota (). These new findings have pointed to less drastic dietary manipulations that could be combined with pharmacological interventions to improve health and prevent disease during aging.
The effectors of dietary restriction, including timing of food intake, specific nutrients, especially proteins and particular amino acids, the gut microbiome and longer-term mechanisms including developmental programming and transgenerational effects, modulate key mechanisms associated with healthy aging.
Meal Frequency and Timing
Only recently have humans and domesticated animals had constant access to food. During evolution, many animals and humans ate only intermittently. For many microorganisms and invertebrates, long periods of starvation are normal and, correspondingly, many of them, including C. elegans, have evolved forms of quiescence in response to the onset of food shortage. Many of the genes that control quiescence are also important in the control of lifespan (Baugh, 2013). Interestingly, intermittent fasting (IF), with alternation of 2-days of ad libitum feeding with 2-day fasting, also extends worm lifespan, through a mechanism involving the small GTP-ase RHEB-1 and insulin/Igf signaling (Honjoh et al., 2009). Even chronic starvation extends lifespan in C. elegans (Kaeberlein et al., 2006; Lee et al., 2006), through mechanisms that overlap with those mediating the response to IF and that include the combined activity of FOXO, FOXA and AP-1 transcription factors in two parallel starvationresponsive pathways (Uno et al., 2013).
In rodents, both fasting for 24 hours every other day or twice weekly extends lifespan up to 30%, independently of both total food intake and weight loss (Mattson et al., 2014). As for chronic DR, the magnitude of the life extension induced by IF can be influenced by the age of initiation and mouse genotype. In A/J mice, for example, IF started at 6 months did not increase lifespan, and when started at 10 months of age reduced mean lifespan by 15% (Goodrick et al., 1990), although it is not clear whether a more or less severe form of IF could extend lifespan in these mice. IF also protects against obesity, cardiovascular disease, hypertension, diabetes, neurodegeneration and the clinical progression of several neurodegenerative diseases (Mattson et al., 2014). In contrast, while many studies report a protective effect against cancer progression (Berrigan et al., 2002; Lee et al., 2012), others suggest detrimental cancer initiation and promotion (Tessitore & Bollito, 1996). In rodents, multiple changes mediate benefits of fasting, including increased production of the neurotrophic factors BDNF and FGF2, reduced inflammation and oxidative stress, and enhanced cellular and molecular adaptive stress responses. Treatment of cells and mice with OHB, an endogenous histone deacetylase inhibitor powerfully induced by fasting, protects against oxidative stress (Shimazu et al., 2013). Fasting improves mitochondrial function, stimulates the production of chaperones such as HSP-70 and GRP-78, and by inhibiting the AKT/mTOR pathway, triggers autophagy and DNA repair pathways in multiple cells types (Brown-Borg HM, Rakoczy S. 2013; Mattson et al., 2014). Moreover, in mice, multiple cycles of fasting modulate hematopoietic stem cell protection, self-renewal, and regeneration via IGF-1 or PKA inhibition (Cheng et al. 2014). Finally, short-term fasting (1-3 days) has been shown to protect rodents against the damage induced by ischemiareperfusion of the liver and kidney by improving insulin sensitivity, reducing expression of markers of inflammation and insulin/insulin-like growth factor-1 signaling, and increasing cytoprotective gene expression (Hine et al., 2014).
Many clinical trials on the effects of IF in humans are underway. A 6-mo randomized clinical trial in overweight or obese premenopausal women showed that fasting for 2 non-consecutive days per week results in reduced body weight, fat mass and waist circumference, and serum concentrations of total and LDL cholesterol, triglycerides, C-reactive protein, and arterial blood pressure. Several serum biomarkers of cancer risk also improve, but total and free IGF-1 do not change (Harvie et al., 2011). Similarly, in 3 small, short-term (8-12 weeks), randomized clinical trial in non-obese and obese individuals, alternate day, 75% fasting lowers body weight and fat mass and risk factors for cardiovascular disease (Kroeger CM, et al., 2014). Preliminary evidence from dogs and humans suggests that short-term fasting (24-48 hrs) prior to chemotherapy reduces some chemotherapy-associated side effects, by protecting normal cells, but not cancer cells, from toxicity (Safdie et al. 2009; Withers et al., 2014).
Patterns of eating over the day can also have substantial effects. Limiting daily food intake of an isocaloric diet to a 5- to 7-h time window in humans can induce health benefits compared with a standard 3 to 5 meals per day (Mattson et al., 2014). Delaying feeding until the evening in diurnally active fruit flies, which normally eat predominantly in the morning, causes an uncoupling of their metabolic cycle from the central circadian rhythm and reduces egg laying (Gill & Panda, 2011). Time-restricted feeding of mice during 8 hours of the dark phase of the daily cycle does not affect overall calorie intake of mice on a high fat diet, but restores normal circadian rhythms of activity in metabolic pathways and protects the mice against weight gain, fat accumulation, inflammation, glucose intolerance, insulin resistance and loss of endurance and motor co-ordination (Chaix et al., 2014). Humans who eat and sleep ~12 hours out of phase from their habitual patterns experience increased blood pressure, worsening of glucose tolerance, a reduction of the satiety hormone leptin and a complete inverse pattern of the cortisol rhythm (Scheer et al. 2009). Although randomized clinical studies on effects of chronic disruption of meal patterns and circadian rhythms, as in shift workers, have yet to be performed, epidemiological data suggest an increased risk of obesity, type 2 diabetes, cardiovascular disease, cancer and neurodegenerative diseases (Wang et al., 2011). Furthermore, 30% calorie restriction by dietary dilution, in which mice ate all day to compensate for the low energy density of the diet, had no beneficial effects on lifespan (Solon-Biet et al., 2013), possibly because of the disrupted meal pattern. Long-lived, hungry DR mice and rats consume their restricted portion of food rapidly, with an extended period of fasting (22-23 hrs) between meals. Chronic DR may hence improve health at least in part through IF. Similarly, in the Wisconsin Rhesus monkey DR trial the animals fed mainly once a day, in the NIA trial twice daily (), which may have contributed to the Wisconsin DR monkeys having a 1.8 times lowered rate of death from any cause (Colman et al., 2014) in contrast with the absence of a difference in death rate in the NIA study (Mattison et al., 2012) (reviewed in Cava & Fontana, 2013). Interestingly, both in overweight/obese and lean women with polycystic ovary syndrome, subjects randomized to earlier meal timing (980 kcal breakfast, 640 kcal lunch and 190 kcal dinner) lost more weight, displayed higher insulin sensitivity, lower serum testosterone concentration, and increased ovulation rate than controls eating isocaloric diets with a later meal pattern (190 kcal breakfast, 640 kcal lunch and 980 kcal dinner) (Jakubowicz et al. 2013).
University of Wisconsin | National Institute of Aging | |
---|---|---|
Rhesus monkeys (n) | 76 (46 m, 30 f) | 120 (60 m, 60 f) |
Genetic origin of monkeys | India | China & India |
Age at baseline | All adult | Juvenile (20 m, 20 f)Adolescent (20 m, 20 f)Old (20 m, 20 f) |
Housing | Single caged | Single caged |
Randomization | 1 CR: 1 Control | 1 CR: 1 Control |
Dietary regimen of CR monkeys | 30% restriction from a BL intake assessed individually | 30% restriction from BL intake levels based on NRC guidelines |
Dietary regimen of control monkeys | Fed ad libitum | Controlled allotment of food each day to avoid obesity (~5-10% CR) |
Meal patterns | Morning meal, plus 100 Kcal integration of food at late afternoon | Twice a day |
Source of nutrients | Semipurified diet rich in refined foods | Natural ingredients (Pesco-vegetarian diet) |
Macronutrient Composition of diets | 15% protein (lactalbumin)10% fat (corn oil)65% carb (cornstarch, sucrose)28.5% from sucrose5% fiber | 17,3% protein (fish, soybean, wheat, corn)5% fat (fish, soy oil, wheat, corn, alfalfa)56.9% carb (ground wheat and corn)3.9% from sucrose5-7% crude fiber |
Vitamin Supplements | Only for CR monkeys | 40% of RDA in Vitamin in both control and CR monkeys |
Food intake measurement | Daily quantification for each animal | 1 week per year |
The molecular mechanisms responsible for the effects of altered meal patters on metabolic health are not fully understood. There may be compensatory changes in energy sensing pathways, such as AMPK, AKT/mTOR, and cyclic AMP response element binding protein (CREB), all implicated in cellular homeostasis and rhythmic oscillations of circadian clock targets (Mattson et al., 2014). Transgenic mice carrying a S714G mutation in hPER1, a circadian clock gene, quickly develop obesity on a high-fat diet (Liu et al., 2014). An isocaloric reduction of fat intake only during the night is sufficient to prevent obesity in these animals (Hatori et al., 2012), suggesting that altered meal pattern alone could be used to prevent certain metabolic diseases (Garaulet et al., 2013; Jakubowicz et al., 2013). Interestingly, a diet high in fat induces a rapid and reversible impairment of BMAL1 recruitment to target chromatin sites, and modifies circadian transcriptome and metabolome profiles (Eckel-Mahan et al. 2013). The profound effects of timing of food intake have hence opened a promising avenue for interventions to improve humans health during aging.
Calories or specific nutrients?
Determining the optimal overall intake and dietary ratios of carbohydrate, fat and protein is challenging. The effects of reduced consumption of a specific macronutrient will depend partly upon how much of it is consumed by the controls and also upon the composition of the rest of the diet. Even for just three macronutrients, the possible combinations are vast. Combinatorial effects can be explored using a geometric framework (see Simpson et al this volume), but this is often not feasible in practice. Dietary composition can also affect overall food intake and its timing, through effects on hedonistic value and satiety. Although such mechanisms are important to understand, they can also complicate analysis of the effects of nutrient intake per se. The effects of diet on health may also be age-specific, a possibility that is starting to be explored experimentally in humans (Wolfe et al., 2008).
Macronutrients
Until recently, reduced intake of calorie rather than of specific macronutrients, was considered important for health benefits of DR. This assumption was primarily based on a flawed interpretation of experimental data showing that 40% calorie-restriction, but not 40% protein restriction, increased lifespan in rats (Maeda et al., 1985). However, the protein-restricted rats were not food restricted, because their growth rate was normal, a point overlooked by the authors of the study. A subsequent series of studies in yeast, invertebrate model organisms and rodents has instead clearly demonstrated that a reduction in specific nutrients in the diet, rather than reduced calorie intake, is primarily responsible for improvements in health and extended lifespan, which is why we use the term DR rather than CR.
Protein and Amino acids
Dietary guidelines from the medical literature and popular press often promulgate high protein intake, especially from animal sources rich in essential amino acids, including sulfur-containing and branched-chain, to combat obesity, sarcopenia, osteoporosis, frailty, surgical stress and mortality. However, accumulating evidence points instead to a restriction of protein or specific amino acids in the diet as promoting healthspan (Grandison et al. 2009; Solon-Biet et al. 2014; Ables et al. 2014; Nakagawa et al., 2012; Pamplona & Barja. 2006). This conclusion initially emerged from investigation of the nutrients mediating the effects of DR, and has since been amplified in broader studies of the effects of dietary composition and intake on health and lifespan.
In Drosophila, restriction of protein-containing yeast, but not carbohydrate or energy, extends lifespan (Mair et al., 2005). Adding back essential amino acids to the diet of DR flies decreases lifespan, with the addition of non-essential amino acids, lipid or carbohydrate exerting little or no effect (Grandison et al., 2009). In mice, also, health and lifespan are strongly affected by the protein component of the diet, with median lifespan progressively increasing up to 30% as the dietary protein-to-carbohydrate ratio is decreased, despite a parallel increase in overall food intake and body fat, and reduction in lean mass (Solon-Biet et al. 2014).
Dietary protein intake is an important regulator of the IGF-1/mTOR network (Efeyan et al, 2012). In humans, unlike rodents, chronic severe calorie restriction does not reduce serum IGF-1 concentration unless protein intake is also reduced (Fontana et al. 2008), suggesting that dietary protein or specific amino acids intake may be as or more important than calorie intake in modulating IGF-related biological processes and disease risk in men and women. In rodents, over-stimulation of the GH/IGF pathway accelerates aging and increases mortality, whereas down-regulation slows aging, prevents cancer and increases lifespan (Junnila et al., 2013). Moreover, serum IGF-1 concentration is inversely correlated with median lifespan in 31, genetically-diverse, inbred mouse strains (Yuan et al., 2009) and with the risk of developing some common cancers in humans (Pollak M. 2012). Interestingly, isocaloric restriction of protein and substitution of plant for animal proteins markedly inhibits prostate and breast cancer growth in human xenograft animal models of neoplasia, with reduced serum IGF-1 levels, and down-regulation of intratumor mTOR activity, histone methyltransferase EZH2 and the associated histone mark H3K27me3, 2 epigenetic markers of prostate cancer progression (Fontana et al., 2013).
Within the protein component of the diet, specific amino acids or their ratio can be important for health and lifespan. Selective restriction of asparagine, glutamate or methionine in the medium has been shown to extend chronological lifespan in yeast (Dilova et al. 2007; Wu et al., 2013a; Wu et al., 2013b). In Drosophila and rodents, restriction of methionine, and in rodents of tryptophan extends average and maximal lifespan (Ables et al. 2014). In the DR Rhesus monkey trials, the Wisconsin diet contained higher concentrations of methionine and branched-chain amino acids derived from lactalbumin than did the NIA diet (), which could explain some of the differences in effects on cancer and mortality.
Amino acid availability is sensed by multiple evolutionarily conserved molecular pathways, the most important being mTOR and GCN2. Activation of mTOR is modulated by different essential amino acid in a tissue-specific manner, with branched chain amino acids playing a key role (Efeyan et al., 2012). In contrast, absence of any individual amino acid activates GCN2, which is required for the protective effect of short-term protein- or tryptophan-deprivation on surgical stress in mice (Peng et al., 2012). The protein/amino acid restriction-induced protective mechanisms against stress, damage accumulation and aging downstream of TOR inhibition and GCN2 activation are unknown. Nonetheless, GCN2 activation stabilizes ATF4, a transcription factor essential for the Integrated Stress Response, which is elevated in several dietary, genetic and pharmacological animal models of longevity (Li et al., 2014; Hine et al., 2014).
Reduced dietary methionine, in particular, induces specific, protective, molecular mechanisms. The sulfur-containing amino acids methionine and cysteine are metabolized through the transsulfuration pathway, lesions in which are associated with increased incidence of age-related pathologies in humans. DR in Drosophila results in increased activity of the rate-limiting enzyme in the transsulphuration pathway, and inhibition of the pathway blocks the response of fly lifespan to DR. Furthermore, transgene-mediated increase in activity of the pathway increases fly lifespan (Kabil et al., 2011). Interestingly, the transsufuration pathway is the primary source of hydrogen sulfide in cells, and hydrogen sulfide can increase lifespan in C. elegans (Miller and Roth, 2007). Furthermore, a transsulfuration-pathway-dependent increase in production of hydrogen sulfide is seen in yeast, worm, fruit fly and rodent models of DR and, in mice, DR-induced resistance against ischemia reperfusion injury requires this increase (Hine et al., 2014). Interestingly, in the long-lived Ames dwarf mouse, which lacks growth hormone, expression of genes in the transsulfuration pathway the flux of methionine to the pathway are increased, associated with higher levels of GSH (Uthus and Brown-Borg, 2006). The lifespan of Ames dwarf mice and of mice lacking the growth hormone receptor is extended relative to controls on normal diet but does not respond to methionine restriction and then becomes similar to that of controls (Brown-Borg et al., 2014). Thus the increased health during aging of these somatotropic mutant mice may be at least in part attributable to increased transsufuration activity.
In humans, little is known on the effects of dietary modifications of protein quantity and quality in modulating molecular pathways that control aging, stress resistance and age-associated diseases. Nonetheless, ongoing clinical trials should soon begin to reveal the metabolic and molecular adaptations induced by protein restriction and alterations of amino acid intake in relativity healthy overweight human subjects and in cancer patients.
Microbiota derived factors and healthy ageing
In humans only ~10% of cells and less than 1% of genes are human, the rest come from trillions of microbes in the gastrointestinal tract. Rapidly accumulating metagenomic data indicate that altered food intake, especially protein and insoluble fiber, have rapid and profound effects on gut microbiota structure, function, and secretion of factors that modulate multiple inflammatory and metabolic pathways (Muegge et al., 2011; Clemente et al. 2012; David et al., 2014; Thorburn et al., 2014). G-protein coupled receptors expressed by enteroendocrine and immune cells may be important mediators of the effects of the microbiome (Thorburn et al., 2014). For example, oral administration to mice of 17, non-pathogenic Clostridia species isolated from healthy human faecal samples results in gut microbiota that provide short-chain fatty acids, bacterial antigens and a TGF--rich environment, which help differentiation, expansion and colonic homing of Treg cells, and reduce disease severity in multiple models of colitis and allergic diarrhea (Atarashi et al., 2013). In contrast, diet-induced microbiota dysbiosis is associated with increased risk of developing cardiovascular disease, obesity-associated metabolic abnormalities, cancer, and autoimmune and allergic disease (Clemente et al., 2012).
In nature, C. elegans feeds on a variety of bacterial species that grow on rotting vegetation, which also constitute the gut microbiome of the worm. Feeding C. elegans with soil bacteria, Bacillus mycoides and Bacillus soli, instead of the standard laboratory E. coli OP50 strain, significantly extends lifespan and stress resistance, suggesting that microbial-derived factors may play modulate pro-longevity pathways (Abada et al. 2009). Moreover, wild-type C. elegans fed respiratory incompetent E. coli show increased lifespan (Saiki et al. 2008). A comparison of the effects of E. coli and Comamonas aquatica on the C. elegans host identified vitamin B12 as a major diffusable factor from Comamonas that influenced patterns of gene expression and the rate of development and fertility of the worm (Watson et al., 2014). Interestingly, effects of chemicals on the worm can also be mediated by the gut microbiome. Metformin, the drug used as the first line of defense against type 2 diabetes, extends lifespan of C. elegans fed on live, but not killed, E. coli, and it does so by disrupting the folate cycle and methionine metabolism of the E. coli. In consequence, the supply of bacterial methionine to the worm is reduced, inducing a type of methionine restriction, consistent with the action of metformin as a DR mimetic (Cabreiro et al., 2013). The relatively simple gut microbiome of Drosophila is also derived from its food intake, and bacterial density and composition has a substantial effect upon the fly host (reviewed in Erkosar and Leulier, 2014). Bacterial density increases during the aging of the fly and can compromise gut integrity (Guo et al., 2014). The normal complement of gut bacteria enables the flies to use low-nutrient or unbalanced diets, by providing them with B vitamins, particularly riboflavin, and by promoting protein nutrition (Wong et al., 2014).
Alterations in the gut microbiome may contribute to the improvement in health from DR and time-restricted feeding. Long-term dietary restriction of mice, with either a normal or a high fat diet, leads to alterations in composition of the gut microbiome, although any contribution of these changes to the health of the DR mice remains to be elucidated (Zhang et al., 2013). In mice on a high fat diet, time-restricted feeding during 8 hours of the dark phase decreases representation of Lactobacillus species, which protect against metabolic disease associated with obesity, and increases Ruminococcaceae species, associated with obesity, are associated with increase is lowered by time restricted feeding (Zarrinpar et al. 2014). Experimental transplantations of microbiota associated with healthy eating could be revealing of their causal role.
Health, disease, longevity, on various timescales including inter-generational
The effects of nutrition, including DR, can be exerted on timescales ranging from more or less instantaneous to inter-generational. For instance, in Drosophila, DR acts acutely, with flies switched between DR and ad libitum feeding almost immediately adopting the mortality pattern of a control group kept permanently in the feeding regime that the switched flies join (Mair et al., 2003). At least as reflected in their mortality rate, these flies thus have no memory of their nutritional history, and their patterns of gene expression also change rapidly in response to DR (Whitaker et al., 2014). In contrast, the mortality rates of C. elegans subjected to one form of DR retain a permanent memory of the previous feeding regime after a dietary switch (Wu et al., 2009). The mortality rates of mice and rats subjected to switches between DR and AL feeding later in life have shown mixed responses, but a meta-analysis suggests that there is a permanent memory of diet in these animals (Simons et al., 2013). Similarly, even short episodes of DR early in adulthood in male mice can induce a glycemic memory apparent as increased glucose tolerance (Cameron et al., 2012; Selman and Hempenstall, 2012). However, in mice and humans, acute responses to DR also occur, including improved insulin sensitivity, reduced inflammation, and protection against ischemia reperfusion injury and other surgical stressors (Hine et al., 2014).
Developmental programming
In contrast to immediate effects of diet, in mammals, including humans, nutrition in early life, including in utero, can have lasting effects on health during ageing, often referred to as developmental programming. For instance, undernourished rat and mouse mothers produce offspring with low birth weight and multiple metabolic defects, including early life adiposity, altered pancreatic function and progressive glucose intolerance (Tarry-Adkins and Ozanne, 2014; Vickers, 2014). Maternal effects on offspring can include changes to the composition of the egg, alterations to the environment in utero, and peri-natal effects such as transmission of the microbiome and alterations to lactation, and can be manifest in the offspring as changes in gene expression and epigenetic modifications including DNA methylation, histone modification and expression of microRNAs, as well as evidence of increased cellular ageing (Aiken and Ozanne, 2014; Colaneri et al., 2013; Radford et al., 2012). Epidemiological data from humans also show a consistent effect of developmental programming by early, including in utero, nutrition, although the evidence on the mechanisms involved is necessarily correlational, rather than experimental. The thrifty phenotype hypothesis (Hales and Barker, 2001) postulates that many of the changes in organ structure and metabolism seen in humans in response to restricted nutrition, particularly of protein, in utero can be understood as the consequences of immediate responses of the fetus to ensure survival and spare vital organs such as the brain. Viewed in this way, an under-nourished fetus makes the best of a bad job, with adverse consequences for health in later life, including reduced glucose tolerance and a higher incidence of ischaemic heart disease, problems that are greatly exacerbated by subsequent adequate or over-nutrition. However, a poor functional capacity for insulin secretion would not be detrimental to individuals who continued to be poorly nourished and remained thin and, therefore, insulin-sensitive, and it remains possible that some fetal and post-natal responses to low nutrition are advantageous in conditions of continuing poor nutrition.
Inter-generational effects of diet
Current nutrition may act as a predictor of future nutritional conditions, if food availability shows local variation, or if timing of natural cycles of food scarcity and abundance occur on an appropriate timescale. Under these circumstances, information gained early in life or even in earlier generations, could be profitably used to anticipate future nutritional prospects and adjust physiology accordingly (Rando, 2012). Such considerations may partly explain why intergenerational effects of diet can also be transmitted through males. The evidence for a role of epigenetic inheritance in these cases is largely correlational, and a direct experimental testing of the hypothesis is challenging (Heard and Martienssen, 2014; Rando, 2012). Cellular mechanisms by which metabolic changes can be communicated to chromatin are being increasingly discussed (Gut and Verdin, 2013; Katada et al., 2012; Lu and Thompson, 2012).
In Drosophila, the sugar content of the paternal diet, even over a 2-day period during which the offspring are sired, can elicit increased lipid content in offspring. Sugar in the diet de-silences chromatin state-defined domains both in mature sperm and in offspring embryos, and H3K9/K27me3-dependent reprogramming of metabolic genes in two time windows in the germ line and the zygote are required for increased lipid content of offspring. Furthermore, data from mice and humans, including discordant human monozygotic twins, show a similar signature of chromatin de-repression associated with obesity (Ost et al., Cell 2014).
Livers of offspring of male mice fed a low protein diet show elevated expression of genes involved in lipid and cholesterol biosynthesis. These alterations are accompanied by subtle (in the region of 20%) changes in DNA cytosine methylation in specific gene regions, including a putative enhancer for the lipid regulator Ppara (Carone et al., 2010). Mice subjected to in utero undernourishment are glucose intolerant, and they can transmit the glucose intolerance, even though they themselves are not undernourished. They experience the effects of maternal undernourishment during a period including the time when their germ cell DNA reacquires methylation. The sperm DNA of these males is hypomethylated at multiple sites, especially ones enriched in regulatory elements and regulators of chromatin. Although these altered methylation patterns are not apparent in the tissues of their offspring, there are perturbations to gene expression, possibly attributable to other types of epigenetic alteration (Radford et al., 2014).
Evidence is also starting to point to truly intergenerational effects of diet, where information about dietary history is epigenetically transmitted in the germ line in the absence of any further input from the organism or its environment. For instance, in the nematode worm Caenorhabditis elegans, starvation-induced developmental arrest has effects that persist for at least 3 generations, with the third generation offspring of the starved great grandparents showing increased adult lifespan. The starvation event leads to the generation of small RNAs that are also inherited for at least 3 generations and that target the mRNAs of genes involved in nutrient reservoir activity (Rechavi et al., 2014), and that are possibly also causal in the increased lifespan of the third generation descendants. It is at present not clear whether these more persistent effects of diet represent non-adaptive perturbations to physiology, anticipatory programming of an adaptive response to nutritional circumstances, or both. Effects of nutrition of the paternal grandfather on grandchildren have been reported in humans, but the mechanisms responsible are unknown (Pembrey et al., 2014). Recent work with mice has suggested that sex-of-parent-of-origin effects may be much more pervasive and influential that previously supposed. Even though the number of imprinted genes in the mammalian genome is predicted to be small, non-imprinted genes can regulate the tissue-specific expression of many other genes differently when transmitted by females or males, possibly by physical interaction with imprinted loci (Mott et al., 2014). These findings could have profound implications for human aging and disease.
Genetic variation in response to diet
Individuals of different genotypes can respond differently to diet. Although little studied outside the context of inborn errors of metabolism, such genetic effects in humans are potentially important for identifying sub-groups that would benefit from dietary modulation. Females and males often respond very differently to dietary and pharmacological interventions, and evidence is mounting for the importance of other types of genetic variation.
DR has proved to extend lifespan in most species examined, including many non-model organisms, although it has been suggested that increased lifespan in response to DR may have evolved in part as an artifact of laboratory culture (Nakagawa et al., 2014). However, strains of C. elegans and Drosophila collected directly from nature respond normally to DR (Metaxakis et al., 2014). Wild-derived mice, on the other hand, can show little or no response (Harper et al., 2006). The stresses of captivity in non-domesticated animals could be part of the explanation for this finding. In addition, wild mice may respond to milder or stronger DR than the single level used in the investigation (Gems et al., 2002). A similar experimental approach applied to recombinant inbred mouse strains, using a single, 40% reduction in overall intake, showed a range of responses from a 98% extension of lifespan to a 68% reduction (Liao et al., 2010). A wider range of reductions intake would have revealed whether these strains differ only in the intake level at which their lifespan peaks under DR, or whether indeed DR does not extend lifespan in some strains. In general, lifespan of model organisms shows a tent-shaped response to the level of food intake, with peak lifespan at intermediate food intake, and a decline through starvation to the left and through increased levels of food intake to the right (Partridge et al., 2005). In order to determine if a strain or species responds to DR, a range of degrees of food intake should therefore be explored (Gems et al., 2002). The apparent lack of response of lifespan to DR trials in the NIA rhesus monkey trial may have been at least in part attributable to the 10% DR applied to the control animals, which may have been sufficient to bring them close to peak lifespan.
Experimental analysis of genetic effects on the response to DR has tended to focus on major genetic variation, caused by either specific gene mutants or inbreeding. While this approach can be informative about mechanisms of the normal response to DR and hence candidates for disease prevention in humans, it may not reveal much about the effects of natural genetic variation in outbred human populations. It is becoming clear that such variation is important. For instance, interventions to reduce weight often have beneficial effects on blood lipid profiles, type 2 diabetes and risk of cardiovascular disease, but some individuals respond poorly or not at all, thus limiting the effectiveness and increasing the cost of intervention programs. A study of the effects on blood lipid profiles and diabetes of increased exercise and lowered intake of fat revealed that a higher genetic risk score for dyslipidemia based upon SNP genotyping was associated with a substantially diminished response to intervention (Pollin et al., 2012). Studies of this kind could also throw light on underlying mechanisms, and hence specific dietary and other types of intervention that could be effective in preventing disease.